The role of condensed tannins in ruminant animal production: advances, limitations and future directions
Click to download the original PDF file!
Tannins represent one of the most abundant polyphenolic compounds in plants. Tannins exist as a multitude of chemically unique entities in nature. The most commonly occurring tannins are typically divided into two major classes based on chemical structure: hydrolysable (HT) or condensed tannins (CT). Hydrolysable tannins are esters of gallic or ellagic acid linked to a polyol core, typically glucose. Condensed tannins or proanthocyanidins consist of flavan-3-ol subunits linked together to form oligomers and polymers. Both HT and CT are defined as astringent, medium-to-high-molecular weight polyphenolic compounds that characteristically bind and precipitate soluble proteins. The objective of this paper was to present recent advances in CT-ruminant interactions, the limitations associated with understanding and using CT in ruminant animal production, and future needs for research to further advance our knowledge of the role of CT in optimization of ruminant animal production. Condensed tannins pose some anti-nutritional problems to ruminants due to their astringent property that reduces feed intake and, consequently, animal performance. Ruminants can, however, tolerate CT by slowly adapting the ruminal microbes to the toxic effects of CT and by releasing CT-binding salivary proteins. The protein-binding ability of CT has some benefits to the ruminant due to complexes formed with essential amino acids, preventing their degradation in the rumen, but releasing them in the lower gut for absorption by the animal. Recent data have suggested increased N retention when CT is given to growing animals. There are potential benefits of using CT and HT for anthelmintic purposes due to their ability to inhibit egg hatching and larval motility of gastrointestinal nematode parasites, especially in small ruminants. Condensed tannins also bind to minerals (Al, Ca, Co, Cu, Fe, Mg, Mn, P, and Zn). Although studies with ruminants have been contradictory, it has been reported that because the CT-metal ion complex is stable over a wide pH range, CT may reduce the bioavailability of minerals. Methane mitigation by feeding CT might be the most impactful benefit for ruminant production. Many empirical equations have been developed to predict ruminal methane emissions, but very few have included CT. Future research should focus on the improvement of methodology to assess CT biological activity, interaction with other plant-specialized metabolites, and associated physiological and nutritional impacts on ruminants.
Key Words: anthelmintic; bypass protein; hydrolysable tannin; methane; polyphenol; proanthocyanidin
INTRODUCTION
Tannins are a subclass of plant polyphenols, which are distinguished from other polyphenols by their ability to form complexes with and precipitate proteins (Hagerman and Butler, 1978; Hagerman, 2012). The commonly occurring tannins are traditionally broadly divided into two categories: hydrolysable (HT) and condensed tannins (CT), whose structures are distinctly different. Although tannins have been characterized predominately on the basis of their ability to bind proteins, reports dating back to as early as the 1930s describe tannins on the basis of “their astringent taste and for their many precipitation reactions with lime, lead acetate, alkaloids, gelatin, albumin, and other proteins, and also for their color reactions with iron salts” (Maitland et al., 1936). Many of these interactions with tannins and organic compounds or trace elements are still of interest today in agricultural research, especially where their potential impact on animal production is concerned. This is likely due to the many challenges and limitations associated with definitively answering questions related to the interactions of tannins in animal physiology and nutrition. It has become evident that furthering our understanding of using tannins in animal production is going to require a better understanding of the role of tannin chemistry in animal interactions. Perhaps this was evident in the 1920s, when Freudenberg put forth a classification scheme for tannins including HT, CT, and an unclassified group of tannins (Maitland et al., 1936), the latter suggesting the existence of tannin structures that were not well understood.
Chemical diversity of tannins
Hydrolysable tannins are typically comprised of a polyol core molecule, usually glucose, but other core molecules can include glucitol, hammamelose, shikimic acid, quinic acid, and quercitol (Hagerman, 2011), whose hydroxyl group of the core polyols have been esterified with gallic acid. Although assembled from relatively simple components, the complexity of HT increases through addition of subsequent galloyl groups, intramolecular oxidative coupling of the galloyl groups of the substituted polyol core, ring opening of glucose core, and oligomerization of the resulting entities through intermolecular oxidative coupling. Based on the structural features arising from these chemical transformations, HT can be divided into three major subclasses: galloglucoses, gallotannins, and ellagitannins. Galloglucoses are glucose molecules in which at least one glucose hydroxyl group has been esterified with gallic acid. A common representative of this subclass is 1,2,3,4,6-pentagalloglucose (PGG) (Figure 1). Gallic acid units can be added to the existing galloyl groups of galloglucoses, which gives rise to the gallotannins. The classic example of a gallotannin is tannic acid (Figure 1). Intramolecular oxidative coupling dimerizes gallic acid substituents forming ellagic acid moieties. This coupling can occur between adjacent gallic acid such as for the conversion of PGG to tellimagrandin II through oxidative coupling of galloyl ester functionalities on the glucose C-4 and C-6 positions of PGG (Figure 1). In turn, casuarictin can be formed from tellimagrandin II through oxidative coupling of galloyl ester functionalities on C-2 and C-3. Opening of the glucose ring followed by additional oxidative couplings give rise to castalagin. These oxidative couplings can also take place intermolecularly, such as with the formation of lambertianin C, a trimer of casuarictin, through coupling of the galloyl groups at C-1 of the glucose core with the C-4/C-6 ellagic acid substituent.
Condensed tannins, also referred to as proanthocyanidins, consist of oligomers or polymers of flavan-3-ol subunits. The flavan-3-ol subunits found in CT varies, but commonly occurring ones include catechin, epicatechin, gallocatechin, and epigallocatechin (Figure 2). Flavan-3-ols differ in their hydroxyl substitution patterns and the relative stereochemistry of the C-2 and C-3 C-ring substituents (circled in Figure 2). Catechin and epicatechin have the same hydroxylation pattern (phenolic substituents on C-5 and C-7 of the A-ring, C-3 and C-4 of the B-ring, and an alcohol substituent in the C-3 carbon of the C-ring). The only difference is the relative orientation in space of the substituent attached to the C-3 of the C-ring. Regarding this relationship, if the C-2/C-3 substituents of the C-ring are represented by both dashed bonds or by both wedged bonds, the substituents are in the cis configuration. If one bond is represented as a dashed bond and one represented as a wedged bond, the substituents are in the trans configuration. When there are two or more chiral (asymmetric) centers, as dashed and wedged bonds (Figure 2), and only one of them is inverted (changed from wedge to dash or vice versa) from a pair of structures which otherwise are identical, the isomer is referred to as an epimer. Thus, catechin with the inverted carbon center at C-3 of the C-ring is referred to as epicatechin. Gallocatechin and epigallocatechin differ from catechin and epicatechin by the inclusion of an additional phenol substituent on the B-ring (indicated by the arrows in Figure 2). This substitution pattern on an aromatic ring, with three phenolic substituents on adjacent carbons, is referred to as a gallo substitution. Thus, with this substitution pattern, catechin becomes gallocatechin and epicatechin becomes epigallocatechin. Catechin and epicatechin are referred to as procyanidin (PC) subunits because, upon oxidation, both give rise to cyanidin. Similarly, gallocatechin and epigallocatechin are referred to as prodelphinidin (PD) subunits because, upon oxidation, both give rise to delphinidin.
Additional flavan-3-ol subunits are present in CT from other plant materials, but occur less frequently than PC and PD subunits. These flavan-3-ol subunits differ in the number of hydroxyl (OH) groups they contain versus PC and PD subunits (Figure 2). These compounds also occur as the epimeric isomers (not shown).
The assemblage of flavan-3-ol subunits into CT oligomers and polymers can occur via several types of covalent bonding patterns (Figure 3). The most common occurring bonding patterns include covalent linkages from the C-4 position of the C-ring of one flavan-3-ol to the C-8 carbon of the subsequent flavan-3-ol subunit or the C-4 position of the C-ring of one flavan-3-ol to the C-6 carbon of the subsequent flavan-3-ol subunit. These linkages are referred to as B-type linkages and are specifically labeled 4→8 or 4→6 B-type linkages. A third relatively prevalent interflavan linkage involves formation of two covalent bonds between participating flavan-3-ol subunits. For example, in addition to an existing 4→8 B-type linkage, an additional covalent bond is formed from the C-7 oxygen atom, from the carbon next to the participation C-8 carbon to the C-2 carbon of the 4→8 connected flavan-3 -ol subunit, and is referred to as a 4→8, 2→O→7 bonding pattern and commonly referred to as A-type interflavan linkages. A-type linkages can be found in CT isolated from cranberries (Foo et al., 2000), peanut skins (Lou et al., 1999), and cinnamon (Anderson et al., 2004).
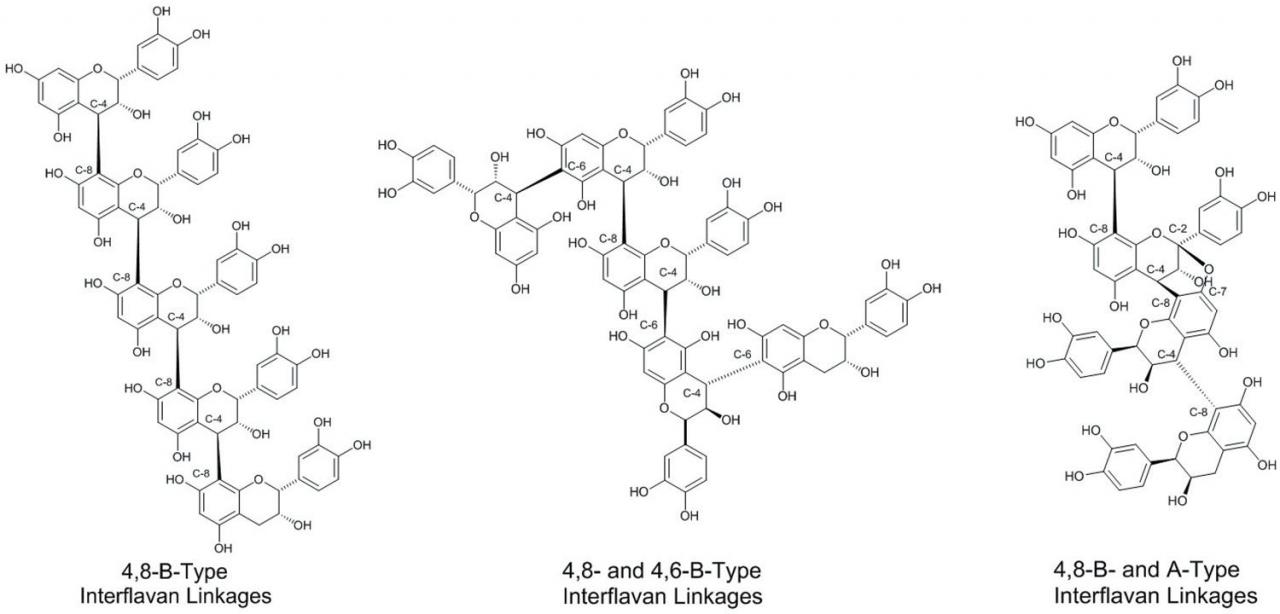
Figure 3 Example of different types of interflavan linkages occurring in condensed tannin oligomers and polymers.
Hydrolysable tannins are believed to be toxic to livestock, including ruminants. Doce et al. (2013) confirmed this by feeding cattle the leaves of Quercus pyrenaica, a genus known to be rich in HT, and reported a marked inhibition of digestion and symptoms of acute toxicity primarily when conditions of feed restriction and malnutrition were prevalent. This may be due to greater protein binding activity of some HT as compared with some CT (Jayanegara et al., 2015). Given the fact that HT (e.g. punicalagin) have hepatotoxic and nephrotoxic effects on some livestock (Filippich et al., 1991), CT are often the focus of research associated with tannin-animal interactions. Nonetheless, future applications of HT as antiviral and antimicrobial compounds (Buzzini et al., 2008) are still a topic of interest for many ruminant nutritionists.
Not all plant species produce CT; and among those that do, concentration and chemical characteristics are highly variable. Some forage plants, such as legumes that are rich in CT, are also generally rich in nutritive value (e.g. protein). For animals that consume CT-containing legumes, perhaps the high nutritive value of the legume helps to counteract the often-observed anti-nutritional effects of CT when animals consume high concentrations of biologically active forms.
The anti-nutritional effect of condensed tannins
Although it is frequently overlooked or disregarded, the fact that much of the biologically active CT-producing plants demonstrate anti-nutritional effects on animals that consume them cannot be ignored. However, generalizations regarding anti-nutritional effects of CT on animals are common. Examples of these generalizations include decreased diet palatability, depressed intake at dietary CT concentrations exceeding 5% of dry matter (DM), depressed digestibility of nutrients (protein, carbohydrates, and fats), and depressed feed efficiency and production of animal products. Mueller-Harvey (2006) provided some much-needed clarification and context for better understanding these anti-nutritional responses.
Palatability is often based on astringency associated with CT-protein complexes formed from proteins in saliva; thus, the greater the protein bound by CT, the greater the astringency and the lower the palatability. However, not all CT bind protein equally. For example, Desmodium paniculatum produces a greater concentration of CT that demonstrate lower protein binding as compared with Neptunia lutea or Lespedeza cuneata that produce lower concentrations of CT with more protein-binding activity (Naumann et al., 2014b). This may explain why Onobrychus viciifolia containing 9-10% CT was more palatable to sheep than Lotus corniculatus, which contain much lower concentrations of CT at 2.6-4% (Haring et al., 2008). Intake is, at least initially, related to palatability. It is possible that intake may be depressed at concentrations less than 5% of DM when the CT are more effective at protein binding and at concentrations greater than 5% DM when the CT are less effective.
Observed decreases in nutrient digestibility by CT are inconsistent. For example, Dalea purpurea with CT concentrations ranging from 6-9% DM had no negative impact on in vivo crude protein (CP) or DM digestibility (Jin et al., 2012). However, Jayanegara et al. (2015) observed decreases in in vivo organic matter (OM) digestibility when CT from different sources and with different chemical structures were added to the substrate. Theodoridou et al. (2010) observed depressed ruminal protein digestibility and a shift from urinary N excretion to fecal N excretion while maintaining body-N retention in sheep fed O. viciifolia containing CT at concentrations of 2.5-3.4% DM. In vivo or in situ studies such as this one mentioned above provide insight into and a greater understanding of the complexity of the relationship between CT and animal nutrition.
The fact that many of the plants that produce CT also produce other plant specialized metabolites cannot be overlooked. It is common to measure CT in the plant or diet and observe animal responses. However, there may be other metabolites that go unmeasured that are responsible for the observed response. For example, Acacia angustissima is a legume that produces a moderate concentration of CT (7-9% DM) and suppresses ruminal methane production (Naumann et al., 2013b), binds protein (Naumann et al., 2014b), and inhibits larval migration of L3 Haemonchus contortus (Naumann et al., 2014a). Condensed tannins may be the only one, of the many plant specialized metabolites found in A. angustissima, affecting these responses (McSweeney et al., 2008). These compounds, individually or in combination, could potentially overwhelm the ability of the animal to detoxify. However, if animals and their rumen microbial populations are allowed to adapt to A. angustissima, symptoms commonly associated with toxicity from this plant are dampened or avoided (Odenyo et al., 1997).
Microbial adaptation to CT is one of the key protective mechanisms for the ability of animals to avoid antinutritional effects. The mechanism by which microbial adaptation protects the animal is unclear, but may be related to shifts in population towards microbes that have the ability to alter the CT (Smith et al., 2005). Goats fed leaves from Quercus semicarpifolia and inoculated with a live culture of Streptococcus gallolyticus, a tannindegrading microorganism, experienced greater DM and CP digestibility, ADG, and feed efficiency as compared with uninoculated goats (Kumar et al., 2014). A shift in rumen microbial population towards Prevotella spp. was observed in Sika deer (Cervus Nippon) fed leaves from Quercus spp., suggesting that either conditions changed in the rumen that resulted in promoting Prevotella or that Prevotella may be able to degrade Quercus tannins (Li et al., 2013). The tannins in Quercus spp. are generally HT rather than CT. However, S. gallolyticus also demonstrates resistance to Acacia CT (≤ 4%) in vitro, whereas S. bovis is inhibited at >0.5% (O’Donovan and Brooker, 2001). Whether S. gallolyticus or other rumen microbes have the ability to degrade CT in the rumen is unclear. More research is needed to better understand how CT affects rumen microbial populations and conversely how CT are affected by rumen populations.
Another key protective mechanism for the ability of animals to tolerate anti-nutritional effects of CT is the production of tannin-binding proteins in animal saliva. Proline-rich proteins have long been identified as an important component in saliva for binding dietary CT (McArthur et al., 1995) and HT (Cappai et al., 2013). Recent studies have indicated that CT do not only interact with acidic and glycosylated proline-rich salivary proteins, but also with histatins and statherins (Soares et al., 2011; Soares et al., 2012b). The aforementioned research was conducted using saliva of human source. Little research has focused on saliva of ruminant livestock. It is unclear as to which types of proteins other than those that are proline-rich occur in ruminant livestock. Mole et al. (1990) determined the proline concentration in saliva of cow, pig, and sheep sources and reported that cow saliva had the greatest proline concentration, but all demonstrated low affinity for tannin. Alonso-Diaz et al. (2012) reported the amino acid profiles of saliva of sheep and goats and found that proline was a minor component relative to histidine, arginine, and glutamate. In addition, affinities of the salivary proteins to different sources of CT differed between sheep and goats, possibly reflecting differences in feeding strategies. It is also possible that the tanninbinding capacity of salivary proteins is a temporary physiological adaptation of ruminants to conserve nitrogen and its production increases only when animals consume diets rich in CT (Vargas-Magana et al., 2013). To further complicate this matter, other dietary constituents, such as carbohydrates, may inhibit the ability of CT to complex with salivary proteins (Soares et al., 2012a). We are starting to better understand the complexity of salivary tannin-binding protein physiology, but targeted studies are still needed to determine the families of tannin-binding salivary proteins present in livestock, namely sheep, goats, and cattle, and to unveil the associated CT binding affinities and inhibitions by other dietary constituents.
Interactions between condensed tannins and protein in the diet
A vast number of studies has documented CT-protein complexation and precipitation (Hagerman, 2012). However, due to the complex nature of the entities involved in these studies, much is yet to be interpreted regarding the control and extent of contribution that both the CT and protein make in the formation of complexes and subsequent precipitation. There is a consensus among tannin researchers that both hydrogen bonding and hydrophobic interactions between the CT and protein play an important role in the complexation and precipitation process. Although the depiction of the hydrogen bonding possibilities between these participants can be conceptualized (Figure 4), the intricacies of intermolecular hydrophobic interactions are less apparent. We have learned that, among the many factors affecting tanninprotein binding (Ozdal et al., 2013), the formation of the CT-protein complex and subsequent precipitate formation depends on the structure of both the protein (Hagerman and Robbins, 1993; de Freitas and Mateus, 2002; Lorenz et al., 2014) and the CT (Poncet-Legrand et al., 2006; Lorenz et al., 2014) under examination, the isoelectric point (pI) of the protein (Hagerman and Butler, 1981; McNabb et al., 1998), the pH of the study medium (McNabb et al., 1998; Adamczyk et al., 2012), and the tannin-protein molar ratios (Hagerman et al., 1998; Charlton et al., 2002; Adamczyk et al., 2012). Experimental evidence suggests that tannins bind to proteins, forming a tannin coating of the protein through a surface adsorption mechanism and this can lead to precipitation of the tannin-protein complex (Dobreva et al., 2011). With high tannin-to-protein ratio, the protein is coated in tannin and leads to its precipitation, whereas a low tannin-to-protein ratio encourages interlinking of soluble tannin/protein complexes, promoting aggregation of the complexes (Spencer et al., 1988; Le Bourvellec and Renard, 2012) and, ultimately resulting in precipitation of the complex.
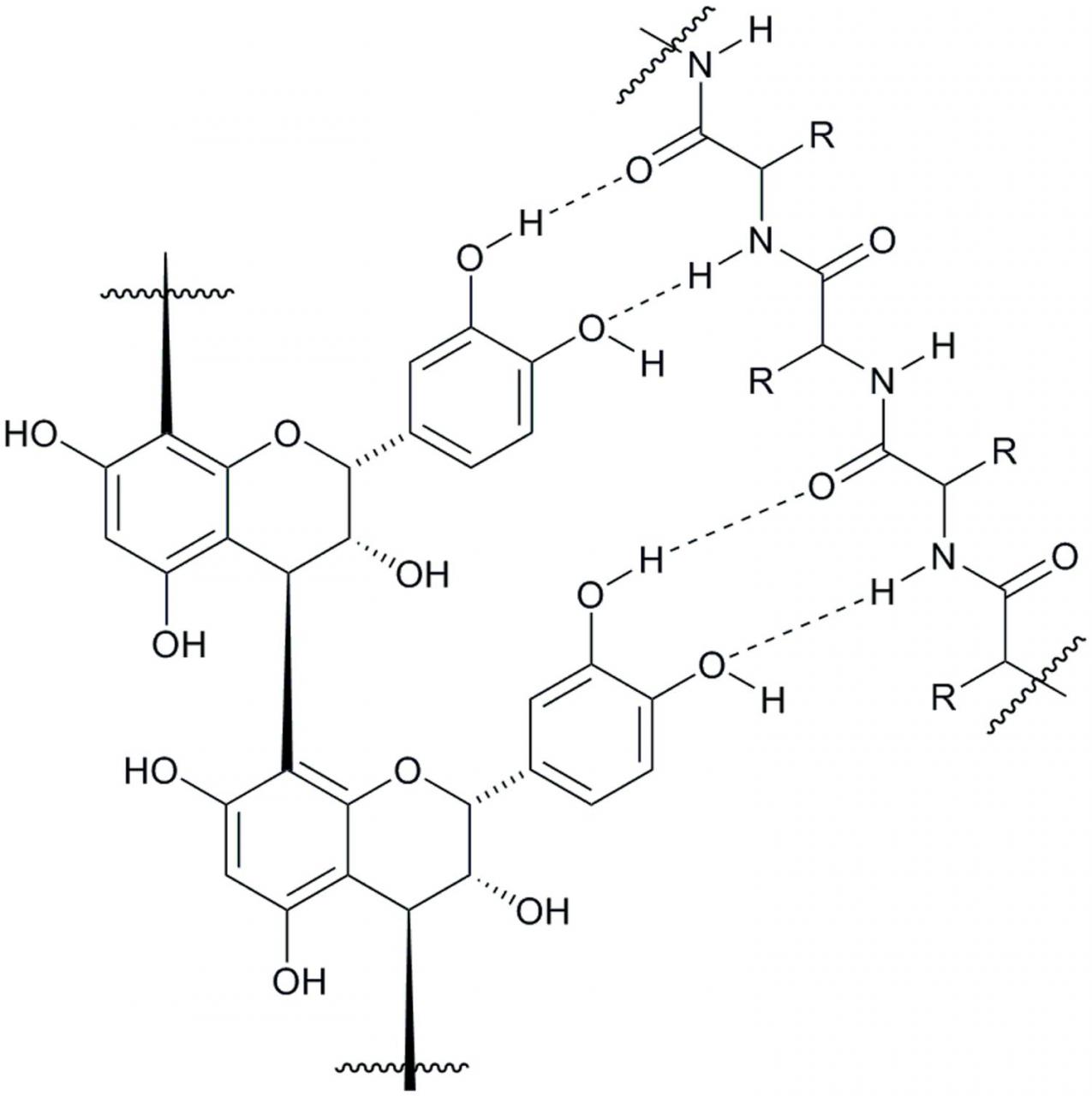
Figure 4 Schematic representation of a hydrogen-bonding array between condensed tannin and a protein amide backbone.
The efficiency of CT-protein binding is highly variable (Hagerman and Butler, 1981; Canon et al., 2011). Once thought to be a non-specific interaction, results reported by Perez-Gregorio et al. (2014) suggest that tannin-protein binding is highly specific. However, specificity of this interaction seems to also be a function of the polarity of the tannin as well as the protein (Perez-Gregorio et al., 2014). The implications of this specificity in ruminant nutrition are complicated by the variation in concentrations and structural characteristics of the predominant proteins and CT present in the rumen (Lorenz et al., 2014) as well as rumen pH, the latter also affected by diet. Dietary constituents may be highly variable in protein types and subsequent amino acid composition, as well as in structural chemistry and types of CT present, resulting in difficulty to predict a particular animal response from CT-protein interactions. Tedeschi et al. (2014) indicated that modeling is one way to understand the complexity of CT associations in the gastrointestinal tract.
To optimize production efficiency, rumen degradable protein (RDP) must be balanced with energy availability to maximize microbial protein synthesis (Brooks et al., 2012). Post-ruminal amino acid composition can be manipulated through supplying rumen-undegradable protein (RUP) to match the amino acid requirements of the animal (Merchen and Titgemeyer, 1992; Schwab, 1995). Common dietary N sources for ruminant diets, such as Medicago sativa and soybean meal, are highly degradable in the rumen, resulting in high ruminal ammonia (NH3) concentrations and low N utilization efficiency – typically around 25% (Calsamiglia et al., 2010). This inefficiency is confounded by high RDP levels that downregulate N recirculation efficiency (Agle et al., 2010) and increase blood urea N (BUN) concentrations, which are associated with reproductive and fertility problems (Elrod and Butler, 1993; Westwood et al., 2000; Roche, 2006; Tshuma et al., 2014).
Condensed tannins may offer an effective strategy to protect dietary protein from degradation through formation of stable complexes in the rumen environment (Patra and Saxena, 2011). The effects of CT from various forage legumes on RDP are well studied, but the relationship is blurred by inconsistent experimental methodologies. In general, inclusion of dietary CT shifts the site of N metabolism and absorption by reducing both the rate and extent of ruminal protein degradation (Coblentz and Grabber, 2013) and NH3 concentrations (McNabb et al., 1996; Min et al., 2005; Agle et al., 2010), increasing postruminal amino acid flow (Waghorn et al., 1994b).
Results from our research team (Naumann et al., 2014c) suggested that we can use a screening tool to predict the potential of structurally diverse mixtures of CT to bind protein based on the concentration of protein-precipitable polyphenols (Figure 5). The linear-segmented regression is represented by Equation 1:
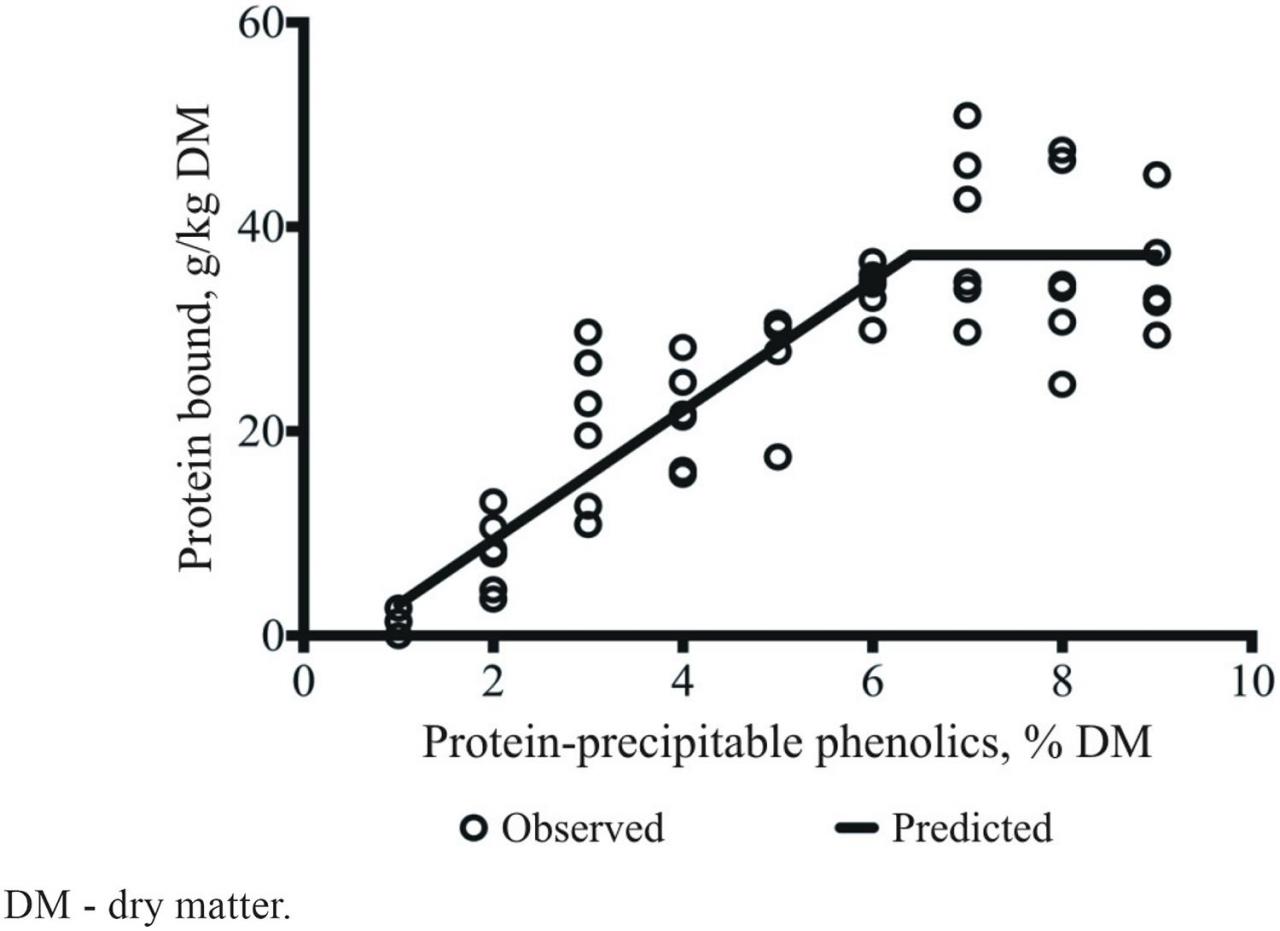
Figure 5 Linear segmented regression of protein bound (g/kg dry matter) on different concentrations of chemically diverse mixtures of protein-precipitable polyphenols (%) on a dry matter basis. Adapted from Naumann et al. (2014c).